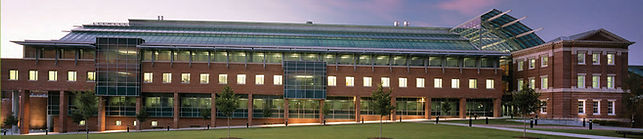


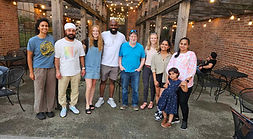

Controlling Lifetime of Biomaterials by Embedding Enzymes
Conventional thinking when designing bioresorbable materials is to use the intrinsic properties of synthesized materials to regulate their degradation rate. For example, considerable effort has been dedicated to introducing comonomers to manipulate the bioresorption rate of PCL1. While this is a well-established strategy, each copolymer composition represents a new medical material that must be assessed by an array of expensive tests requiring years. Herein, we describe a simple but powerful alternative approach to control biomaterial lifetime using PCL as the model system. The hypothesis tested is that, biomaterials bioresorption rate can be regulated by embedding an enzyme within a bioresorbable polymer matrix when that enzyme is active for matrix hydrolysis. The PCL-degrading enzyme, Candida antarctica Lipase B (CALB), was selected and surfactant paired to render CALB soluble for dispersion within a PCL matrix. This principle termed embedded enzymatic degradation can be used for any biomaterial for which an enzyme is known or can be developed for matrix hydrolysis. Therefore, it represents a general approach to be deployed to control biomaterial lifetime.
PCL is an excellent candidate for controlled release and other biomaterial applications due to its availability, biodegradability, ease by which it can be melt-processed into various shaped substances, biocompatibility and miscibility with many drugs2. PCL is a soft material that has a peak melting temperature, tensile stress at break, elongation at break and tensile modulus of 60 oC, 4 Mpa, 800-1000 % and 386 Mpa, respectively3. However, numerous studies have shown that PCL degrades slowly when implanted in animals. For example, PCL capsules, implanted in rats, with initial molecular weight (Mw) 66 000, retained their shape for over 2-years2. By 30 months, PCL capsules broke into low molecular weight pieces (Mw 8000)2. The slow degradability of PCL limits its use for systems that require shorter lifetimes1. Nevertheless, PCL and its copolymers continue to be intensely studied for a range of medical applications.
Herein, surfactant-paired CALB was mixed in toluene with PCL and films were prepared by solution casting. Sodium bis(2-ethylhexyl)sulfosuccinate (AOT), the surfactant used to solubilize CALB, is approved for administration to both humans and animals5 and was found to be safe for encapsulation and delivery of lipophilic drugs in aqueous based delivery systems6,7. Biocompatibility experiments of CALB are currently under progress. If CALB biocompatibility is not suitable, methods are available to identify and remove segments in proteins that trigger an immune response8. Another alternative currently under study is exploring other enzymes that have high homology to those naturally found in animal systems and that also catalyze PCL hydrolysis.
CALB was surfactant paired with AOT to enable enzyme transfer from the aqueous to the organic phase. The method of ion-pairing is described in supporting information. Ion-paired CALB was embedded in PCL matrices with enzyme contents of 6.5 and 1.65% (w/w). Methods in the supporting information describe details by which PCL embedded CALB films were incubated during degradation studies. Weight loss was determined gravimetrically and protein content was quantified using the micro BCA protein assay. Molecular weights were determined at room temperature by gel permeation chromatography (GPC). Scanning electron micrographs were generated with an Amray 1910 SEM and Schottkey field emission gun at 5 kV. DSC measurements were performed using a Perkin-Elmer DSC system with a scan rate of 10 oC/min. CALB was conjugated with fluorescein isothiocyanate (FITC) and this conjugate was embedded in PCL matrices. Imaging of CALB distribution within PCL was performed by confocal microscopy (Leica Microsystems, Bannockburn, IL) using a 488 nm excitation laser.
References
-
Ganesh, M.; Dave, R.N.; L’Amoreaux, W.; Gross, R.A. “Embedded Enzymatic Biomaterial Degradation” Macromolecules, 42 (18), pp 6836–6839 (2009).
-
Little, U.; Buchanan, F.; Harkin-Jones, E.; McCaigue, M.; Farrar, D.; Dickson, G. Polymer Degradation and Stability 2009, 94, 213-220.
-
Sun, H.; Mei, L.; Song, C.; Cui, X.; Wang, P. Biomaterials 2005, 27, 1735-1740.
-
Gross, R. A.; Kalra, B. Science 2002, 297, 803-807.
-
Bolgen, N.; Vargel, I.; Korkusuz, P.; Y, Z. M.; Piskin, E. Journal of biomedical materials research. Part B, Applied biomaterials 2006, 81B, 530-543.
-
Barnhart, E. R. 1989, 49.
-
Liu, J.-G.; Xing, J.-M.; Chang, T.-S.; Liu, H.-Z. Bioprocess and Biosystems Engineering 2006, 28, 267-273.
-
Gupta, S.; Moulik, S. P. Journal of Pharmaceutical Sciences 2008, 97, 22-45.
-
Sojin Shikano, B. C., Haiyan Sun and Min Li. Nature Cell Biology 2005, 7, 985 - 992.
-
Nisida, H., and Y. Tokiwa. . Journal of Environmental Polymer Degradation 1993, 1, 227 - 233.